PART 2
What do we mean by “highly coherent, usually monochromatic beam of electromagnetic waves”?
For the sake of brevity, from now on we will use the word ‘light’ to denote not only visible light (electromagnetic waves our eyes can see) but electromagnetic waves in general. That is, when we say ‘light’ we mean electromagnetic waves, including radio waves, gamma rays and ultraviolet.
Now, a monochromatic ray of light is one in which the waves have the same wavelength or frequency. (The word ‘monochromatic’ came from the Greek words mono, meaning one, and chromos, meaning color.) A ray of white light is not monochromatic since it consists of waves of different wavelengths. In other words, white light is made up of light of different colors. On the other hand, the light emitted by a colored light emitting diode (LED) is usually monochromatic. A blue LED emits only blue light.
The fact that laser beams are highly monochromatic finds use in many scientific and engineering applications, such as spectroscopy. In spectroscopy, laser beams with a very specific wavelength are sent through a sample to be analyzed.
The next important property of laser beams is their high coherence. (What is certain is that they more coherent than the CBCP or the Vatican.) In the language of wave physics, coherence is the property of having waves that oscillate in phase of each other. Coherence comes in two kinds, temporal coherence (coherence in time) and spatial coherence (coherence in space).
Temporal coherence (coherence in time) would be best explained by an analogy in dance: for two dancers to be able dance the tango well, their stepping must have the same tempo. In other words, dancers performing a ballroom dance must have temporally coherent foot movements.
Temporal coherence is very closely related to monochromaticity. In fact, temporal coherence is used to measure monochromaticity. Another important aspect of temporal coherence is uniform polarization. This gives laser beams their characteristic ‘glare’, which makes them dangerous to the eyes. Sometimes, the glare of laser beams is used by the police or the military to disorient a pursued individual or an enemy.
Spatial coherence, on the other hand, means that a ray of laser light can be focused to a very narrow beam, often called a “pencil beam”. In other words, laser light can be focused to a very small spot. This makes laser beams ideal for applications that require great precision, like reading digital information encoded in a CD, cutting intricate patterns into metal or wood, burning away tumors without destroying neighboring healthy cells, or correcting vision problems without further damaging the patient’s eyesight. In microscopy, lasers are used to obtain blur-free images of very small objects at various depths, and this is possible because laser beams can be very narrow. And do not forget the use of lasers in increasing the chance of a headshot.
”]
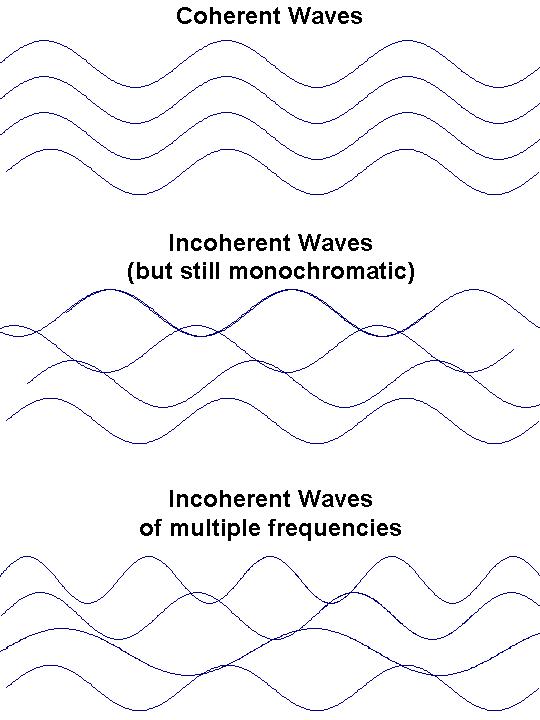
Spatial coherence is also the reason why lasers have high intensity. To understand why, it is important to know what intensity is in physics. Intensity is defined as the power distributed over a given area. In equation form,
Here, power is the energy delivered by a source of light per second. The more energy a source is releasing in a second, the more power it delivers. Power is measured in watts (W). We are all familiar with the fact that different household appliances have different “power ratings”. The higher the power rating of an appliance, the more energy it delivers per second. In the case of light bulbs, a light bulb with greater power rating delivers more light energy per second than another light bulb with a lower power rating. For example, the light energy released per second by 20-W light bulb is two times more than the light energy released by a 10-W light bulb.
But notice that intensity is power over area. If power is distributed over a large area, then the intensity will be low. For this reason, the intensity of light from a normal light bulb dies down quickly as one go away from the source. In a normal light source, light energy is distributed over an area that becomes larger as one goes farther from the source. On the other hand, because of the spatial coherence of light emitted by lasers, the light energy they emit is concentrated in a very small area. This results in a very high intensity beam. The difference between a normal light bulb and a laser is illustrated by Figure 2 below. Figure 2a depicts the light emitted by a normal light source (like the light bulbs used at home). Figure 2b depicts the light emitted by a laser.
Because laser beams are composed of light rays that are concentrated in a very tiny area, they have very great intensity. Any Star Wars fan knows this; in the hands of an evil Empire, the high intensity of lasers can be used to wipe out whole races and destroy entire planets in a single colorful display of lights (all while orchestra music plays in the background, of course).
Back to the real world, the great intensity of laser beams finds countless industrial applications such as in laser cutting, laser wielding, laser brazing, laser melting and laser bending.
As with almost all technologies, the limits of laser technology depend only in the imagination of the engineer. In the hands of a very creating engineer (hopefully not an engineer of the Empire), the applications of lasers is limitless. Other current laser applications are laser ranging (using lasers to measure great distances), pollution monitoring, therapeutic skin treatments, holography, and nuclear fusion (where lasers are used to compress a nuclear fuel tight enough to cause a fusion).
And can you still imagine using a ball mouse? I can’t. So in summary: lasers rule!
We are now ready to address the last question: how are such beams of electromagnetic radiation produced in the first place? In the last part of this series we will see how.
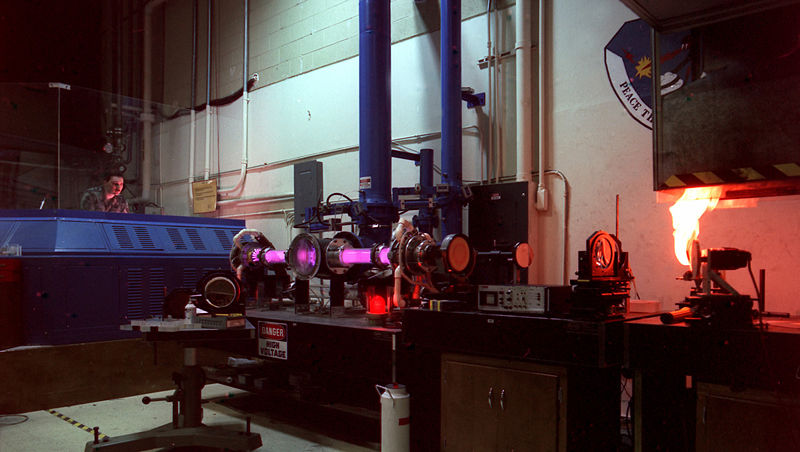
PART 3: How are laser beams produced?
References:
[1] Hitz, Ewing and Hecht, Introduction to Laser Technology, 3rd ed. IEEE Press, 1998.
[2] Griffiths, Introduction to Electrodynamics. Prentice-Hall, 1999.
[3] Harris, Nonclassical Physics. Addison-Wesley, 1999.
[4] www.knowledgerush.com